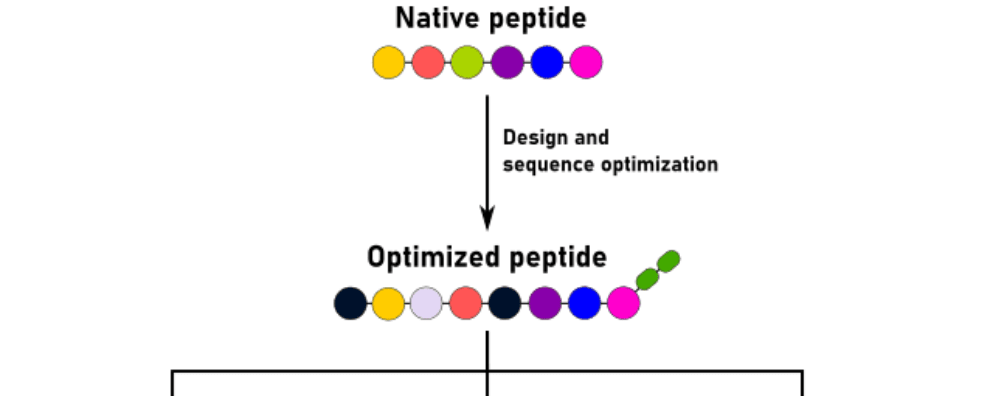
Challenges in chemical and recombinant peptide production processes
In peptide production, there’s no one-size-fits-all solution. Chemical and microbial recombinant production technologies are recurrently used and combined in an attempt to maximize production yields and increase the purity of the final product. In this article, we will address some of the most common challenges associated with large-scale peptide production, as well as discuss which solutions scientists propose to overcome them.
- Challenges associated with synthetic peptide production
- Striving for sustainability in synthetic peptide production
- The development of microbial recombinant peptide production processes
- Challenges in recombinant peptide production methods
- Solutions to overcome the limitations in recombinant peptide production
- Concluding remarks
Challenges associated with synthetic peptide production
A successful peptide production strategy starts with a good peptide design and optimization. Classical approaches to peptide production include liquid-phase and solid-phase synthesis. These two approaches, alone or in combination, can be used to generate high-quality peptides for most applications.
However, peptide complexity for therapeutic applications has been steadily increasing for the past decade. Today, many of these peptides contain more than 30 amino acid residues, which can make the synthesis process more challenging from a chemical point of view.
One approach that is recurrently being used to overcome this limitation is the convergent peptide production approach.
This approach allows the chemical synthesis of long peptides by performing the chemical ligation of smaller fragments. Kemp and colleagues from MIT (USA) have pioneered this technique in the late 1970s. The further optimization of this technique resulted in the development of the native chemical ligation (NCL) method which is still in use today.
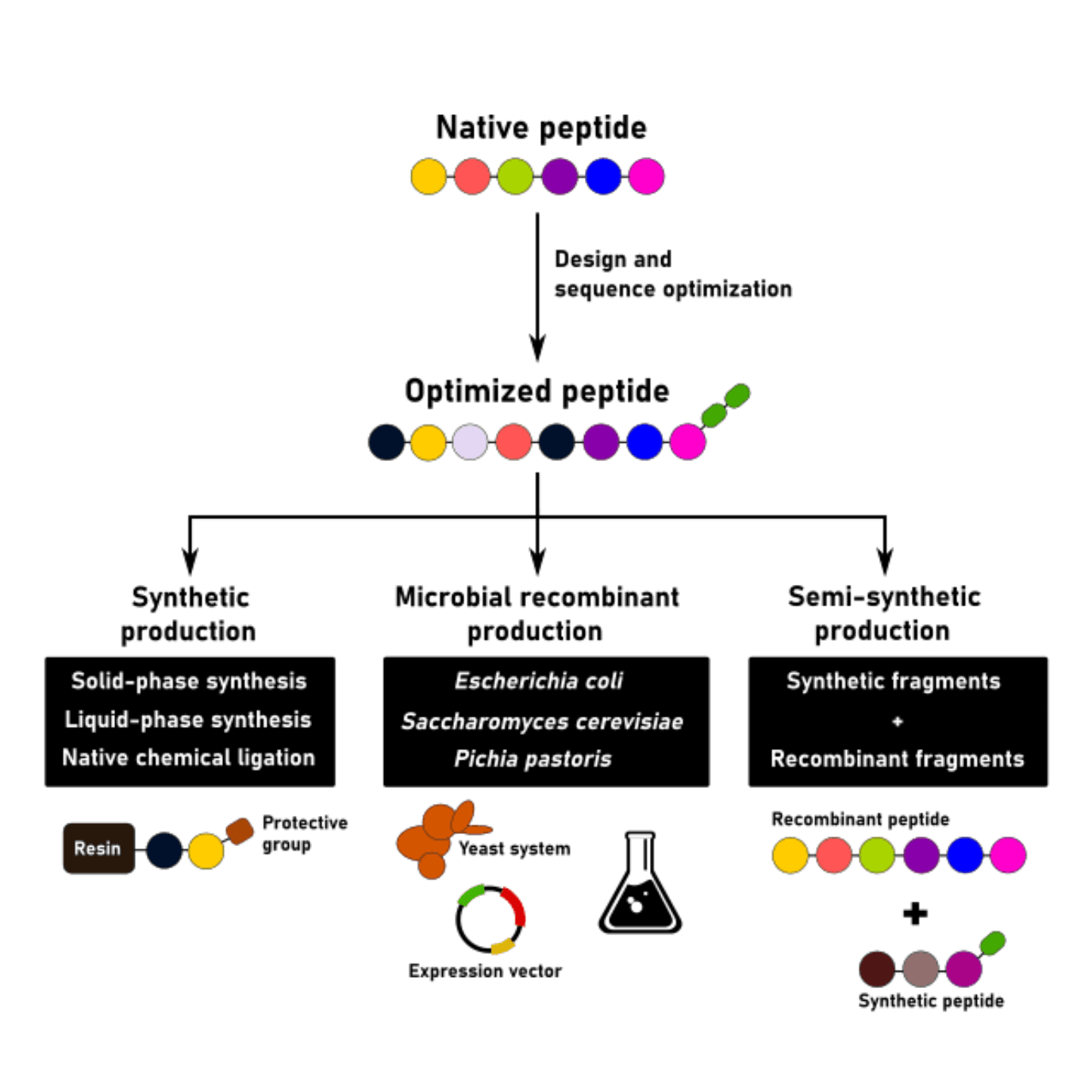
This method allows the coupling of the N-terminal of one peptide to the C-terminal of another peptide while maintaining the native sequence of the fragments intact. An advantage of this technique is the high stability of the starting products and the ease of establishing peptide bonds between them.
Moreover, NCL has allowed getting the most out of liquid-phase and solid-phase synthetic processes for the production of long peptides such as collagen-like polymers and lipopeptide–carbohydrate vaccines, among others. Besides, most ligation reactions can be performed in aqueous solutions with very high yields and purity levels.
But, even today, more than 60 years after the discovery of solid-phase synthesis, peptide production still faces many challenges:
- Low yields for some peptides due to insolubility issues
- Toxic byproducts generated by the need to use strong solvents and hazardous reagents during the synthetic process
- High purification costs for longer peptides, that are often contaminated with byproducts and trifluoroacetic acid (TFA), which is usually used to cleave the peptides from solid-phase resins
These limitations continue to propel the development of green chemistry-based approaches.
Striving for sustainability in synthetic peptide production
Major sustainability issues related to peptide synthesis have been raised in the past decade. These issues relate to the use of strong solvents, hazardous coupling agents and difficulties in recycling resins used both in peptide synthesis and chemical ligation.
One of the greatest challenges in peptide synthesis is the development of sustainable and efficient catalytic methods. Solid-phase peptide synthesis depends on the efficient formation of amide bonds between amino acids. Currently, most amide coupling reagents are benzotriazole derivates such as uranium/aminium salts (e.g. HATU, HCTU, TBTU) or phosphonium salts (PyBOP, PyAOP, and PyClock). These traditional reagents enhance coupling efficiency and reduce the frequency of amino acid racemization.
But these reagents are also known to possess explosive properties, making the work at high temperatures dangerous and increasing the difficulty of scaling-up these chemical methods.
Another major issue with peptide production through solid-phase synthesis is the use of strong solvents. Solvents represent the biggest portion of wastes generated by chemical synthesis. Two of the most valid approaches to solve this issue include the use of environmental-friendly solvents, and/or the development of more effective solvent-recycling processes.
The most common reagents in solid-phase synthesis are dimethylformamide (DMF), N-methyl-2-pyrrolidone (NMP), and in lower amounts, dichloromethane, diethyl ether, and tert-butyl methyl ether.
All of these solvents present several hazards. More importantly, DMF and NMP, the two most commonly used solvents, present reproductive toxicity hazards and, thus, their use are likely to be restricted by the European Regulation for Registration, Evaluation, Authorization, and Restriction of Chemicals (REACH).
For these reasons, many researchers have proposed developing solid-phase peptide synthesis in water. This would eliminate hazardous solvent usage. Some progress has been made in this area. For instance, several water-soluble protecting groups have been described recently such as sulfonated versions of Fmoc and Boc protecting groups.
Moreover, hybrid approaches have been proposed to perform the coupling and deprotection step in both organic and aqueous solvents and, thus, mitigating the environmental burden of this process and minimizing the frequency of the side-reactions associated with aqueous synthesis.
The development of microbial recombinant peptide production processes
In the past decade, peptides approved for therapeutic applications tend to be longer and, thus, more difficult to produce. In industrial settings, the synthesis of peptides with up to 40 to 50 amino acids can be achieved by chemical methods with acceptable yields. But peptides with ≥ 20 amino acids are already considered challenging to produce using these methods alone.
In these cases, several researchers have proposed harnessing recombinant technologies for peptide production. The first peptide ever to be produced by recombinant processes was insulin. This peptide, with 51 amino acid residues, paved the path for recombinant peptide production in the modern-day industry.
After its approval, many other peptides produced using recombinant and semi-synthetic methods have been approved. The latter harnesses the advantages of chemical and recombinant production by performing the ligation of synthetic peptide fragments with recombinantly produced peptides.
A few examples of therapeutic peptides produced through recombinant processes include:
- Insulin – recombinant production approved in 1991, contains 51 amino acids
- Calcitonin – recombinant production approved in 2005, contains 32 amino acids
- Ecallantide – recombinant production approved in 2009, contains 60 amino acids
- Teduglutide – recombinant production approved in 2012, contains 33 amino acids
It is estimated that around 20% of the peptides currently in preclinical and clinical trials are currently produced using recombinant or semi-synthetic technologies. And these numbers are expected to increase in the coming decades.
Several classes of emerging peptides are currently preferably produced using recombinant technologies. These classes include antimicrobial peptides (AMP) and toxins derived from animal venoms which were found to have applications for therapy, cosmetics, and insecticides.
Challenges in recombinant peptide production methods
Despite their lighter environmental burdens and lower production costs, recombinant methods are still considered more complex and labor-intensive than their synthetic counterparts. Chemical methods take less time to develop in comparison to recombinant methods, which makes the former more amenable to fast scale-up and quick production of peptides for preclinical and clinical trials.
Moreover, recombinant production is restricted to peptides containing natural amino acids alone. A limitation that can be circumvented by performing the ligation of synthetic fragments to the N or C-terminal of the native peptide.
The most common expression vectors for recombinant peptide production include Escherichia coli, Saccharomyces cerevisiae, and Pichia pastoris. More complex systems, such as mammalian (e.g. CHO or HEK) and insect cell lines are generally avoided due to their high costs, lengthy development process and difficulties in scaling-up the production. Moreover, most peptides with commercial value do not need to undergo complex post-translational modifications to become functional.
For these reasons, peptide synthesis by recombinant methods is usually reserved for high-performing microbial expression systems.
But there’s no universally suitable vector for peptide production. Bacterial expression vectors are generally associated with higher downstream production costs because these organisms do not secrete the peptide to the culture medium and they produce endotoxins that need to be removed before commercialization.
On the contrary, the use of yeast vectors usually possesses high secretory efficiency, which reduces the cost of downstream purification. However, P. pastoris usually requires methanol to induce gene expression, and thus requires the use of explosion-proofed equipment. Moreover, S. cerevisiae shows high protease activity which can result in degradation of the final product, and lower expression levels when compared to P. pastoris production.
Solutions to overcome the limitations in recombinant peptide production
Short peptides can be easily degraded by microbial proteases.
In E. coli this issue can be addressed using two different approaches: use of protease deficient strains, peptide production as insoluble inclusion bodies or as concatemers.
The production of peptides as insoluble inclusion bodies serves a double function. It protects the peptides from protease activity and the expression host from the toxic effects of the peptides. Besides, this strategy increases the yield of bacterial production, as peptides are mostly pure inside inclusion bodies and they can easily be separated from all other cell components.
The greatest challenge associated with this strategy is the need for strong denaturating reagents to resolubilize peptides and the need for a subsequent refolding step to recover bioactivity. Hence, this strategy is more suitable for the expression of short peptides with no complex secondary structures.
Interestingly, it was proposed as early as 1984 the production of peptides as tandem repeats separated by cleavable residues. This strategy was shown to increase the production yield and protect these peptides from protease activity. The ideal number of multimers ranges from 2 to 15, with 3 being the most common. Moreover, these multimers are commonly fused with molecular tags in vivo to increase their stability.
Common tags include His-tag (for affinity purification), thioredoxin (TrxA), small ubiquitin-like modifier (SUMO) and self-cleavable intein tags.
Both TrxA and SUMO are known to enhance the solubility of peptides and thus can be used to increase the production yields of insoluble peptides. Also, the self-cleavable intein-based tags are known to reduce the toxicity and enhance the purification efficiency of toxic peptides. These tags can self-cleave in the presence of reducing agents or as a response to temperature, salt, and pH changes, thus, reducing the need for expensive tag removal processes.
Concluding remarks
Peptide production can currently be achieved by synthetic and recombinant technologies or a combination of the two. Both approaches present different limitations that can be circumvented using a variety of different methodologies.
However, the greatest problem associated with large-scale peptide production is the identification of the best-performing methodology. It can be hard to predict which methodology will give higher production yields. Thus, the development and evaluation of the best production method can be a lengthy process, entirely dependent on the nature of the peptide.
- Chandrudu, S. et al. Chemical Methods for Peptide and Protein Production. Molecules. 2013 ; 18:4373-4388. doi: 10.3390/molecules18044373
- Commission Regulation (EU) 2018/588 amending Annex XVII to Regulation (EC) No 1907/2006 of the European Parliament and of the Council concerning the Registration, Evaluation, Authorisation and Restriction of Chemicals (REACH) as regards 1-methyl-2-pyrrolidone (2018). Official Journal of the European Union, L 99, pp. 4-6.
- Isidro-Llobet, A. et al. Sustainability Challenges in Peptide Synthesis and Purification: From R&D to Production. J Org Chem. 2019; 84(8):4615-4628. doi: 10.1021/acs.joc.8b03001
- Pennington, M. et al. Peptide therapeutics from venom: Current status and potential. Bioorg Med Chem. 2018; 26(10):2738-2758. doi: 10.1016/j.bmc.2017.09.029
- Wegmuller, S. and Schmid, S. Recombinant Peptide Production in Microbial Cells. Curr Org Chem. 2014; 18(8):1005-1019. doi: 10.2174/138527281808140616160013
- Zhou, L. et al. TrxA mediating fusion expression of antimicrobial peptide CM4 from multiple joined genes in Escherichia coli. Protein Expr Purif. 2009; 64(2):225-230. doi: 10.1016/j.pep.2008.11.006